Most people, both the population at large and those in healthcare technology management (HTM), are familiar with certain large and complex machines inside hospitals and clinics, such as MRIs, CT scanners, and PET scanners. There is one machine, however, that most people have not heard of, and often those in HTM aren’t even familiar with the department that uses it. I’m talking about medical linear accelerators and the radiation oncology department. Radiation oncology is often confused with radiology, though as you’ll soon see, they are very different.
Often hidden deep in the basement, linear accelerators (linacs) and radiation oncology are more specialized than the other radiology systems and departments mentioned above. You may have an MRI scan for any number of reasons, but with very little exception, you’ll only learn what a linac is if you or someone close to you has cancer that needs radiation therapy. What’s more, linacs are also often referred to as simply, “the machine,” like that life-sucking device from The Princess Bride, so many people don’t know what a linac is even after they’ve been treated on one.
Radiation oncology works a bit differently than most other departments. So many departments like to say, “things work a little differently here,” and as BMETs we smile and nod. But radiation oncology truly is a different beast. For example, it is one of the only departments that can’t perform clinically if its network goes down. You can still take an MRI, and you can still perform surgery, but you can’t treat patients with a linac if there’s no connection to the server.
Also, there are many steps along the way to treatment, involving many professionals with various disciplines. There is no one person who takes care of a patient. In fact, nurses and medical doctors play a relatively small role in the patient’s therapy.
There are six clinical subdepartments within radiation oncology:
- Simulation;
- Dosimetry;
- Physics;
- Nursing;
- Doctors; and
- Therapists
And we’ll see how they work together to develop a course of action to treat a patient.
This article will explain how the department works, including not just the role the linac plays in treatment, but how every facet of the department comes together to treat a patient. This is important to understand, in addition to knowing how the machine works, in order to be a competent service professional.
Simulation
After the initial consultation with the doctor, this is the first stop on the road to treatment. The name “simulation” can be a bit misleading. It would be a little more appropriate to call it something like “tumor locating,” or maybe “patient coordinates.” Simulation is where the patient comes to have the precise location of the tumor identified and recorded. Most often the simulators are CT scanners themselves, though MRI/CT fusion is becoming more popular.
Of course, the tumor is already identified prior to this stage using diagnostic imaging, but the importance of being scanned in a simulator is that it has a coordinate system, isocenter, and tabletop matched to the linac the patient will be treated on. For a good end result in treatment, you must start knowing exactly where the patient is in relation to the CT scanner, and where the CT isocenter is in relation to the linac isocenter. Any mistakes made here will result in errors propagating down the line.
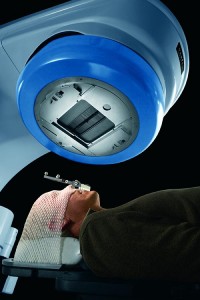
Patient’s head is immobilized using a plastic mask, and the jaw is positioned with a bite block. All photos courtesy Varian Medical Systems, except where otherwise indicated.
The simulation techs place the patient on the table, scan them, and mark the tumor location in 3D on the patient’s skin using permanent tattoo ink—basically, tattooing a crosshair onto the patient. Motion must be minimized to increase accuracy. To this end, patients are immobilized, sometimes even sacrificing their comfort to achieve the necessary accuracy.
To immobilize the head, a mask is placed over the patient’s face and attached to the table, and heat-molded to create a custom fit for that patient. Various other devices are used to hold the patient in the same, repeatable position, and will be transferred from the simulator to their treatment machine. For example, a mat filled with expanding foam, similar to that used in shipping goods, can be put under the patient, expanding around the patient to create a custom mold of their body.
Similarly, a device very much like a bean-bag mattress, on which the patient lies in the treatment position, has all its air removed and the beans vacuum-lock into the shape of the patient. Whatever devices are used, the goal is the same—to locate the tumor with the patient in a specific position, then to put them back into that same exact position for their treatments.
Even with all this immobilizing, the tumor can still move inside the patient. For example, lung tumors can move with breathing, and prostate tumors can move with urine level in the bladder. Tumor motion inside of the body can be detected with 4D scans, and can be accounted for during treatment by means of specific treatment techniques.
Dosimetry
Also known as “treatment planning,” this is something of the central nervous system for the department. It’s where the patient’s treatment plan is developed. Many factors are taken into account when developing a treatment plan, such as the following:
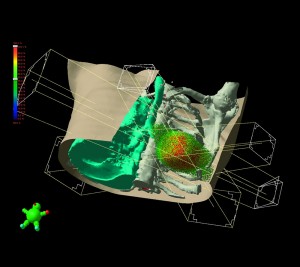
Demonstrating a treatment plan for lung cancer, avoiding spinal cord and opposite lung through multiple beam angles. Click to enlarge.
Tumor position. What is the surrounding tissue made of? Fat, muscle, lung, organ, etc, all respond differently to radiation, and some things, such as nerve cells, can’t repair much radiation damage at all. Since tumors have a tendency to spread, doctors like—when possible—to irradiate the area immediately surrounding a tumor as well as the tumor itself.
Sensitive structures. If something sensitive to radiation is in the beam path while trying to hit the tumor, it could suffer massive damage.
Tumor depth. How deep or superficial a tumor is located changes what energy is used. A deeper tumor requires a more penetrating energy.
Type and progression. Different tumors, categorized by parameters such as location, stage, progression, and more, take varying amounts of radiation to eradicate. Since radiation is toxic and the damage is irreparable, doctors always try to prescribe the minimum effective dose. However, if too little radiation is prescribed, the tumor might survive, and because the damage doesn’t heal (radiation damage is similar to burns in this regard), radiation will no longer be an option the second time around.
Amount of radiation. If a patient received all the radiation needed to kill a tumor all at once, it’s very possible that it could be fatal to the patient as well. The dose is spread out, with the patient most often receiving one treatment per day over many weeks.
And this is really only the tip of the iceberg—there are still many more factors that must be accounted for, and no two treatment plans are ever the same. The bottom line is that it’s an extremely complex practice that is accounted for as much as possible.
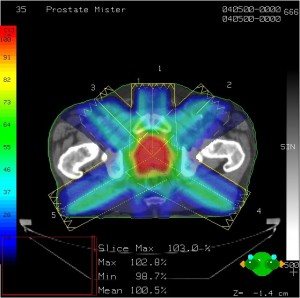
Treatment planning software. Multiple beam angles converge, delivering prescription dose to the tumor while sparing other tissue and structures. Color wash shows intensity of irradiation.
Dosimetrists take the doctor’s prescription (eg, 4,000 centi-Grey of radiation) and use sophisticated CAD-like software to engineer a treatment plan according to the physician’s specifications in a way that won’t harm the patient.
In addition to being spread out over weeks, the dose is also spread out over multiple beam angles, sometimes called “fractions.” Imagine if you took a pencil and lightly drew a line on a piece of paper, then lightly drew another line, and another, and another, all from different angles. The point where they intersect will be much darker than each individual line.
That’s much how radiation therapy works, but instead of pencil lead, it’s radiation. That point of intersection is known as the isocenter, and the patient’s tumor is placed in the machine’s isocenter. The amount of radiation going through any one beam-line isn’t too high, but it hits the isocenter again and again, delivering a lethal dose to the tumor.
Physicists sometimes work with the dosimetrists on especially complex plans. They supply expert knowledge of the machine and translate its capabilities into clinically useful knowledge, so it’s fairly common to see them in dosimetry.
Finally, the physician examines the plan and requests tweaks if needed. The physician is the one who ultimately approves or rejects the treatment plan, and takes full responsibility for the treatment of the patient.
Physics
Physicists play a huge role in radiation oncology, especially from the BMET’s perspective. They’re often the BMET’s primary contact for problems with the linac, and because they take responsibility for the machine performing as designed, many adjustments and calibrations require physicists to either be present, or to check machine functionality afterward.
While doctors take ultimate responsibility for the treatment of a patient, physicists take immediate responsibility for the creation and safety of a treatment plan. To verify that it is safe, they run quality assurance tests on the plan to make certain that the machine mechanics are working as expected, that the right amount of radiation is being delivered, and that everything is generally going as planned. The plan QA is a bit like dress rehearsal before actual therapy.
Where physicists really get their hands full, though, is taking ultimate responsibility for the linear accelerator. Quality assurance of linacs could be a full-time job in and of itself! To make sure they’re adhering to some sort of standard, a group of physicists called the American Association of Physicists in Medicine assigns task groups to determine the best way to test a certain parameter or characteristic of the machine.
The group then publishes the results and recommended procedures. That’s what most physicists follow when testing the machine. For example, they might say, “I’m running TG142 on the MLC tonight,” meaning they’re performing the test Task Group 142 recommended to QA-test the multileaf collimator.
The many types of QA tests that are performed can be lumped into three general categories:
Mechanical movements: Making sure the gantry’s isocenter is still accurate; verifying that when the couch indicates that it moves by 0.3 cm, it actually does; checking positioning and repeatability of the multi-leaf collimator; checking walkout of the collimator, etc.
Radiation output: Making sure what the machine thinks is one centi-Grey of radiation actually is, checking the flatness and symmetry of the treatment beam, verifying that the light field and x-ray field position are coincident, etc.
Image quality: Imaging was not part of radiation therapy for a long time, but these days, imaging a patient while treating them is the standard of care. Whether it’s imaging with a KV tube or using the MV beam, the QA is similar: checking geometric accuracy, testing Hounsfield Unit calibration, creating dead-pixel maps, etc.
Physicists often have to check the machine after a calibration is performed or a part is replaced to make sure it’s still working as expected. Because of this, radiation oncology engineers and physicists often work hand in hand to make sure the machine is running as designed.
Nursing/MDs
From an HTM perspective, nurses and doctors play a relatively minor, though still critical, role in radiation oncology. The doctors, for example, are very much like the quarterback of the team. They may be constantly tossing the ball to other people who take it and run with it, but they’re calling the plays and take responsibility if something doesn’t work out. They decide how much radiation to give a patient, review images during treatment to make sure therapists are still hitting the mark, and check in routinely with the patient to make sure everything is going according to plan.
The nurses are specially trained in problems that can arise due to radiation treatments. Radiation is dangerous, and if not performed correctly, it can result in serious harm to the patient. Even good treatments can cause problems such as skin irritation and burns, nausea, or irritated bowels, depending on what area of the body is being treated. They also are on the lookout for significant problems from an error, such as slurred speech, loss of motor control, or open wounds.
Treatment
I remember a Lean enterprise consultant once using an exercise of breaking a service down to its most basic components in order to see where the real value is. For example, when you get on a plane to fly somewhere, you don’t need the baggage handlers, gate agents, security agents, or anything else that comes along with it. At the most basic level, you just need a plane and a pilot to fly it.
Using that technique, radiation oncology comes down to needing a linac and a radiation therapist to run it. Yes, it would be good to have someone fixing the linac, physicists to check them, doctors to prescribe the right amount of radiation, etc, but the real meat and potatoes of the department are the linac and the therapists.
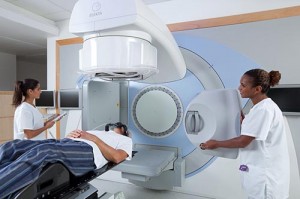
Therapists setting up a patient. Note the x-ray tube and panel, rotated 90 degrees from the treatment head, for KV imaging. Image courtesy of Elekta.
Radiation therapists run the machine to treat patients. They download the plan onto a workstation, and set up the patient according to the plan. This will match exactly the location specified way back in “simulation.” They use the same masks and body-fixation devices created during simulation to put the patient back into the same position, and align the patient’s tattoos to the isocenter of the linac.
These days, they’ll also almost always take 2D-radiograms or a CT to make sure the tumor is still where expected and truly aligned with the linac isocenter. This is especially important for things like prostate cancer, where the patient has to have a full bladder before treatment to force the tumor into the same location every time.
Some machines have kV sources built into them for imaging, and some will mount a whole CT scanner in the vault, moving it over the treatment table to acquire a scan. Almost all machines can also take images, both 2D and 3D, with their megavoltage treatment-beam x-rays, although this generally results in poor image quality compared with kilovoltage x-rays.
Unlike diagnostic-level radiation, where the tech can view the patient through leaded windows, in radiation oncology the patient is separated from the therapists by multiple feet of concrete, lead, steel plate, and a borated polyethelene sandwich. They maintain audio-visual contact with the patient through CCTV cameras and an intercom system.
These systems are in place first for safety—in fact, they can’t treat a patient without them—but they also are used to coach the patient through treatment. They sometimes have to remind the patient to hold still (especially if they’re in an uncomfortable position or their extremities are falling asleep) and when to breathe if the therapy is respiratory-gated. They will offer encouragement when the patient needs it, and, with young patients, will even have parents read their child’s favorite story to them over the intercom.
How Treatment Happens: A Technical Perspective
A patient’s treatment plan is stored on a server—sometimes in the department, sometimes in a hospital data center, or sometimes even at a massive private data center in another part of the country. It is composed of many different sets of information:
Images: Both the images from “simulation” and all the images taken during treatment. Also, the database records any shifts made in patient position based on those images.
Setup information: This includes patient position, machine position, and any accessories used, such as wedges or bolus.
Treatment information: A huge amount of data, this includes whether the treatment is using x-rays or electron beams, what energy level, how much radiation per fraction, collimator shapes, and more.
All of this information is sent to the treatment workstation in a specialized flavor of DICOM called DICOM-RT, specific to radiation oncology. DICOM-RT has sections to include the extra information needed to treat a patient.
The linac produces radiation consisting of either a high-energy electron beam or x-rays. Which type is used to treat a patient is decided when creating the treatment plan. The beam is then collimated using a multileaf collimator (MLC). The MLC is made of anywhere from 52 to 160 leaves, sticking into the radiation beam like fingers. Just as you can make complex shadow-puppet shapes using your fingers in a beam of light, the MLC can form the radiation beam to match the exact size and shape of a tumor.
The output of the machine is monitored by ion chambers in the beam’s path. The machine uses the ion chamber to see how much radiation is delivered, and shuts the beam off after the prescribed dose for that angle is met. In addition to the quantitative dose measurement, it checks the quality of the beam as well, making sure its strength is equal in all areas of the beam.
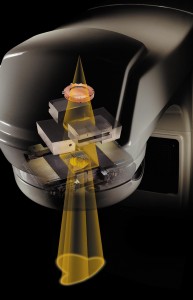
Visible here are the copper-colored ion chamber used to measure output and the jaws and MLC used to collimate and shape the beam.
At the same time, the treatment workstation is working in concert with the machine. It maintains constant contact with the linac to keep giving it new plan information as the treatment progresses. It can also interlock the machine if it detects a problem, and can start and stop the beam as needed for things such as respiratory gating.
As the machine treats the patient, pieces of data such as finished angles, amount of radiation as measured by the ion chambers, and all machine parameters and positions for each fraction are verified and uploaded to the database to be permanently recorded. The software combination of the treatment workstation and database is often called a “verify & record” system. It is a term you’ll see frequently in radiation oncology.
It is done this way so that what was actually delivered can be compared to what was prescribed, and so as the treatment goes along over days and weeks, it can be reviewed to make sure it is going as planned.
All of the data of the V&R system is stored in a database on a separate server. This is sent to the treatment workstation on a per-patient basis, and delivered treatment data is sent from the treatment workstation to the database in close to real-time.
Because of this, the network between the two (usually the hospital network, although sometimes it is all on a private network) plays a vital role. If the hospital network goes down, they’ll no longer be able to treat patients. They won’t be able to download any more treatment plans for patients, and the data from the treatment they’re working on won’t be recorded in the database.
With modern computing power, some manufacturers are starting to work around this by putting a mini-database on the treatment workstation. That way, a day’s worth of patient data can be downloaded from database to workstation all at once, and treatments can be recorded to the mini-database on the workstation and simply be uploaded once the network connection is back.
A treatment typically takes only 15 minutes per day, but this process is then repeated for anywhere from a number of days to a couple of months. At the end of the treatment path, hopefully, a patient will be cancer-free.
More to Learn
I hope this overview of radiation oncology has shed some light on a department that is often unknown to BMETs. I know when I went to school for my AAS in biomedical electronics, the basics of topics such as x-ray, CT, and MRI were broached, but lesser-known modalities such as NM and radiation oncology were not given any mention and were a complete mystery.
This is still simply a general overview and there is always more to learn in this field, but hopefully you now know your way around the department of radiation oncology, and can speak with more understanding and authority when dealing with people from the department. Those of us in the business of repairing medical equipment often have to fight hard for respect from clinicians, and knowledge is our strongest ally when trying to work hand in hand with them.
Andy Kremer, field service engineer and creator of www.clubhtm.com, has more than 12 years experience in biomedical electronics, a decade of which has been in radiation oncology. For more information, contact editorial director John Bethune at [email protected].